User:Debra Tabron/sandbox
Many contaminated sites use active remedies such as pump-and-treat or in situ remediation to clean up impacted groundwater. Natural attenuation processes such as natural degradation or hydrodynamic dispersion also contribute to the cleanup. As remediation progresses, a point is often reached when the time required to reach the remedial objectives using the active remedy is roughly the same as the time required if the active remedy is shut down, and the continuing remediation of the site is provided by natural attenuation processes alone. From that point forward, the extra effort and expense of the active remedy provides no benefit over natural attenuation, and it may be appropriate to transition the site to Monitored Natural Attenuation (MNA). This article deals with currently available tools and approaches that can be used to support a decision to transition from active remediation to MNA.
Related Article(s):
- LNAPL Source Zone Conceptual Models (Coming soon)
- LNAPL Remediation Technologies (Coming soon)
CONTRIBUTOR(S): Andrew Kirkman
Key Resource(s):
- LNAPL Site Management: LCSM Evolution, Decision Process, and Remedial Technologies[1]. Section 3 provides an introductory discussion of LNAPL mobility concepts.
- API LNAPL Distribution and Recovery Model (LDRM), API 4760 [2]. Provides detailed discussion of LNAPL mobility as related to saturation, soil properties and capillary pressure.
- Technical measurement guidance for LNAPL natural source zone depletion[3].
- Section 6 provides a discussion of compositional changes to LNAPL over time and how to use those changes to calculate natural source zone depletion rates.
Introduction
LNAPL can be found in the subsurface in three different states:
- Residual LNAPL is trapped and immobile but can undergo composition change and generate dissolved hydrocarbon plumes and/or vapor sources in the unsaturated zones.
- Mobile LNAPL is LNAPL at greater than the residual saturation which can therefore accumulate in a well where it is potentially recoverable. However, in this state LNAPL saturation is insufficient to drive migration (i.e., the LNAPL body is not expanding).
- Migrating LNAPL is LNAPL at greater than the residual saturation that expands into previously unimpacted locations over time (e.g., LNAPL appears in a monitoring well that had no previous detections).
When released to the subsurface, light non-aqueous phase liquids form source zones which evolve over time with changing impacts to soil, groundwater, and at some sites surface water. Shallow unsaturated soils may also be impacted by intrusion of vapors released by the LNAPL. (See Vapor Intrusion - Separation Distances from Petroleum Sources.) LNAPL mobility is typically highest during the time of the release to the environment. Once the release stops, LNAPL mobility and the potential for migration rapidly decrease. The LNAPL body may initially contain mobile LNAPL near the release point. However, as the LNAPL body spreads, the driving head and pressure gradient decrease, and the LNAPL thickness (a key factor in mobility) decreases. The LNAPL body also begins to weather, losing volatiles and biodegradable compounds. Over time, this weathering process alters the LNAPL composition and stabilizes the LNAPL body, and the fraction of LNAPL that remains mobile therefore decreases[1][3][4]. LNAPL bodies from historical releases are dominated by immobile residual LNAPL.
Because LNAPL bodies evolve over time, LNAPL mobility is a much more significant concern during and immediately after the release period than it is after the release is halted. Tools are now available to assess the magnitude of LNAPL mobility at individual release sites to support decision making regarding potential remedial actions. One such tool is the American Petroleum Institute’s LNAPL Distribution and Recovery Model (LDRM) which is available as a free download. Please see the LNAPL Mobility and Recovery section below for a more complete discussion of modeling tools.
In order to understand LNAPL mobility, key concepts from the science of multiphase flow in porous media (e.g. soil and aquifer material) are important. Consider dry soil to which a small amount of water is added. The open pore space in the soil, initially filled with 100 percent air, now contains a mix of air and water. Therefore, a soil can have more than one fluid within the pores (“multiphase”). The fraction of pore space filled with a given fluid is termed “saturation”. The air saturation of the initially dry soil was 100% and the water saturation was zero. When considering a petroleum release site, three different fluids may exist in the pore space: air, water and LNAPL. The sum of the saturation of the three fluids is 100%. All three fluids can exist within the subsurface with varying saturations.
LNAPL Mobility Conceptualized
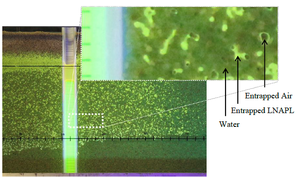
Figure 1 shows a research tank with a well screen in a porous media (sand) containing LNAPL. The LNAPL fluoresces under ultraviolet (UV) light, while water and air do not. The column of yellow fluorescence in the well represents the gauged LNAPL thickness and corresponds to the vertical interval over which LNAPL flows in the formation[6]. The yellow fluorescence to the left of the well represents LNAPL occurring within soil pores. Below the potentiometric surface line, the pores are dominated by water (dark pores) and LNAPL. Above this line LNAPL and water still exist in pores, but the air saturation increases with elevation. The incomplete yellow fluorescence in the formation illustrates how water and LNAPL occupy pores over the mobile interval. This occurs because the mobile LNAPL cannot displace all of the water from the pores.
LNAPL mobility can be described mathematically by Darcy’s Law, an empirically derived equation describing the flow of fluids through porous media where the specific discharge, q, is equal to the product of the hydraulic conductivity, K, and the hydraulic gradient, i. While this is the same Darcy’s Law that is used to describe groundwater flow (see Advection and Groundwater Flow), there is an important additional term, Relative Permeability, that is included when using Darcy’s Law to describe the flow of LNAPL in the subsurface. Relative permeability is the ratio of the effective permeability of a fluid at a specified saturation to the intrinsic permeability of the medium at 100-percent saturation[7]. The USEPA (1996)[8] describes relative permeability this way:
References
- ^ 1.0 1.1 ITRC, 2018. LNAPL Site Management: LCSM evolution, decision process, and remedial technologies (LNAPL-3). Interstate Technical and Regulatory Council
- ^ Charbeneau, R.J., 2007. LNAPL Distribution and Recovery Model. Distribution and Recovery of Petroleum Hydrocarbon Liquids in Porous Media. Vol. 1. API Publication 4760. Report.pdf
- ^ 3.0 3.1 CRC CARE, 2018. Technical measurement guidance for LNAPL natural source zone depletion. Cooperative Research Centre for Contamination Assessment and Remediation of the Environment, Newcastle, Australia. Technical Report no. 44. 254p Report.pdf
- ^ Mahler, N., Sale, T. and Lyverse, M., 2012. A mass balance approach to resolving LNAPL stability. Groundwater, 50(6), pp.861-871. [10.1111/j.1745-6584.2012.00949.x 10.1111/j.1745-6584.2012.00949.x
- ^ Hawkins, A. M., 2013. Processes Controlling the behavior of LNAPLs at groundwater surface water interfaces, Master of Science Thesis, Colorado State University, Ft. Collins, Colorado. Report.pdf
- ^ Huntley, D., 2000. Analytic determination of hydrocarbon transmissivity from baildown tests. Groundwater, 38(1), pp.46-52. doi: 10.1111/j.1745-6584.2000.tb00201.x
- ^ Mercer, J.W. and Cohen, R.M., 1990. A review of immiscible fluids in the subsurface: properties, models, characterization and remediation. Journal of contaminant hydrology, 6(2), pp.107-163. [Mercer, J.W. and Cohen, R.M., 1990. A review of immiscible fluids in the subsurface: properties, models, characterization and remediation. Journal of contaminant hydrology, 6(2), pp.107-163. doi: 10.1016/0169-7722(90)90043-G
- ^ USEPA, 1996. How to effectively recover free product at leaking underground storage sites. A guide for state regulators. USEPA 510-R-96-001. U.S. Environmental Protection Agency, 162 pp. Report.pdf